When it comes to building particle accelerators the credo has always been “bigger, badder, better”. While the Large Hadron Collider (LHC) with its 27 km circumference and €7.5 billion budget is still the largest and most expensive scientific instrument ever built, it’s physics program is slowly coming to an end. In 2027, it will receive the last major upgrade, dubbed the High-Luminosity LHC, which is expected to complete operations in 2038. This may seem like a long time ahead but the scientific community is already thinking about what comes next.
Recently, CERN released an update of the future European strategy for particle physics which includes the feasibility study for a 100 km large Future Circular Collider (FCC). Let’s take a short break and look back into the history of “atom smashers” and the scientific progress they brought along.
A Machine to Split the Atom
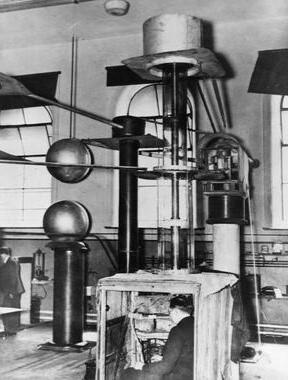
Credit: cambridgephysics.org
The main motivation to build accelerators arose at the beginning of the twentieth century when Ernest Rutherford discovered in 1919 that he could split nitrogen atoms by bombarding them with alpha particles from natural radioactive sources. To continue his research, he demanded a source of higher energy and higher intensity “atomic projectiles” than those provided by natural radioactive sources. Encouraged by Rutherford, in 1932 Cockcroft and Walton used a 400 kV generator to accelerate protons and shoot them onto a lithium target which resulted in the first entirely man-controlled splitting of the atom.
Particle acceleration using DC voltages like that of the Cockroft-Walton generator and later the Van de Graaff generator was limited by the maximum voltage that the machine could provide. To overcome this limitation, Swedish physicist Ising proposed the principle of resonant acceleration where the same voltage is applied repeatedly through a series of drift tubes hooked up to an RF generator. This was considered the true birth of particle accelerators and in fact, the current generation of linear colliders are still relying on the same principle. Rolf Winderöe was the first to build such an accelerator in 1928 in Germany to produce 50 keV potassium ions.
From Linear to Circular
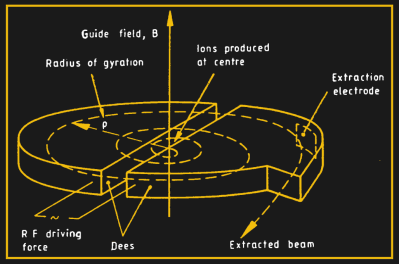
Credit: P.J. Bryant
One downside of the linear accelerator (linac) is that the length of the drift tubes has to be increased as the velocity increases making the machine rather large and difficult to construct for high energies.
In 1929 Ernest Lawrence came up with the much more compact cyclotron, which accelerates particles along a spiral path guided by a magnetic field. Together with his student M. Stanley Livingston, Lawrence built the first cyclotron which was only 4 inches in diameter but could accelerate protons to 1.25 MeV. The cyclotron finally made it possible to produce particles with much higher energies than those by radioactive sources and it stayed the most powerful type of accelerator until another technology came along in the 1950s.
Keeping Particles in Sync
As particles start to approach the speed of light they slow down due to relativistic effects and loose synchronization with the RF electric field of the cyclotron. This was compensated by varying the RF frequency and the machine and was dubbed the synchrocyclotron. Later also the guiding magnetic field was ramped up as the particle velocity increases so that the particles moved on a constant orbit. This was the birth of the synchrotron.
The final advancement was made by moving from fixed target accelerators to storage ring colliders. Since the energy available for the production of new particles is given in the center-of-mass frame of the collision, it is much more efficient to collide particles head-on instead of shooting a beam on a fixed target.
A Plethora of New Particles
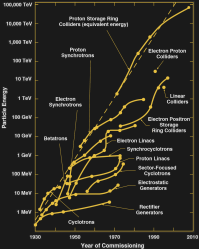
Credit: R. Ruth
While before the 1950s new particles were mainly discovered through cosmic rays, powerful accelerators like the synchrotron heralded a “Golden Era” of particle physics. These new machines led to the discovery of many subatomic particles as listed in the table below.
Studying the structure of the atom on smaller scales and being able to produce particles with higher masses is what drove the development of accelerators with ever-higher energies. In a synchrotron reaching higher energies requires either a larger radius or stronger magnetic fields. Therefore, it was the use of superconducting magnets but also the possibility to build colliders underground, below property that is not owned by the laboratory running the machine, which enabled the construction of giant colliders such as the LHC.
Year | Particle | Accelerator Name | Accelerator Type | Location |
---|---|---|---|---|
1955 | antiproton | Bevatron | proton synchrotron | LBNL, U.S. |
1962 | muon neutrino | AGS | proton synchrotron | BNL, U.S. |
1974 | J/ψ meson | SLAC | electron linac | Fermilab, U.S. |
1975 | tau lepton | SLAC | electron linac | Fermilab, U.S. |
1979 | gluon | DORIS | electron synchrotron | DESY, Germany |
1983 | W, Z bosons | SPS | proton synchrotron | CERN, Switzerland |
1995 | top quark | Tevatron | proton synchrotron | Fermilab, U.S. |
2000 | tau neutrino | Tevatron | proton synchrotron | Fermilab, U.S. |
2012 | Higgs boson | LHC | proton synchrotron | CERN, Switzerland |
What’s Next?
Currently, particle physics is in a bit of a crisis because the final missing piece of the Standard Model, the Higgs boson, was discovered by the LHC but there is yet no evidence for new physics like supersymmetry. Although we know that the Standard Model cannot explain dark matter and dark energy, it is doubtful that a new giant collider such as the FCC will provide any answers which is why some people strongly argue against it. There is no reason for nature to be nice, so it might be that the mass of new particles lies far beyond what is technologically achievable.
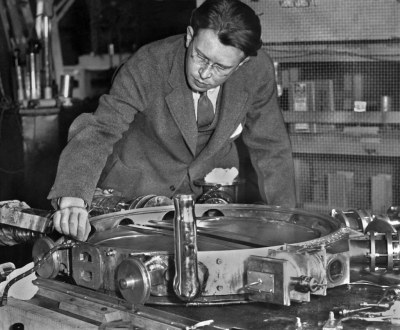
It may also be that new physics is hiding somewhere in the low-energy regime which would require entirely different experiments. Nevertheless, there are some technological developments that may considerably lower the price tag of a new supercollider thereby making it more attractive. One would be the discovery of room-temperature superconductors the other is Wakefield acceleration which could ultimately lead to much more compact accelerators that can even fit on a table (again). So let us hope that pushing the energy frontier will keep providing us answers to the most fundamental questions in nature.
No comments:
Post a Comment